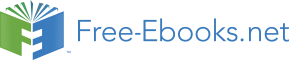

Maintaining intensively manicured athletic turf grass can be a challenge due to many factors: heat, chilling temperatures, drought, humidity, elevated ultraviolet radiation, and abiotic stresses. This book identifies the three key areas that I think are of major importance in the prevention of stress to natural grass athletic playing fields based on my 29 years of experience maintaining intensively manicured natural grass playing fields and maintaining peak performance out of them: protecting and maximizing chloroplast function, improving rubisco function, and preventing and managing oxidative stress(free radical) damage.
The word chloroplast is derived from the Greek word "chloros" meaning "green" and "plastes" meaning "the one who forms". The chloroplasts are cellular organelles of green plants and some eukaryotic organisms. These organelles conduct photosynthesis. They absorb sunlight and convert it into sugar molecules and also produce free energy stored in the form of ATP and NADPH through photosynthesis. Chloroplasts are unique organelles and are said to have originated as endosymbiotic bacteria.
Chloroplast Diagram
Chloroplast Structure
Chloroplasts found in higher plants are generally biconvex or planoconvex shaped. In different plants chloroplasts have different shapes, they vary from spheroid, filamentous saucer-shaped, discoid or ovoid shaped.
They are vesicular and have a colorless center. Some chloroplasts are in shape of club, they have a thin middle zone and the ends are filled with chlorophyll. In algae a single huge chloroplast is seen that appears as a network, a spiral band or a stellate plate.
The size of the chloroplast also varies from species to species and it is constant for a given cell type. In higher plants, the average size of chloroplast is 4-6 µ in diameter and 1-3 µ in thickness.
The chloroplast are double membrane bound organelles and are the site of photosynthesis. The chloroplasts have a system of three membranes: the outer membrane, the inner membrane and the thylakoid system. The outer and the inner membrane of the chloroplast enclose a semi-gel-like fluid known as the stroma. This stroma makes up much of the volume of the chloroplast, the thylakoids system floats in the stroma.
Outer membrane - It is a semi-porous membrane and is permeable to small molecules and ions, which diffuses easily. The outer membrane is not permeable to larger proteins.
Intermembrane Space - It is usually a thin intermembrane space about 10-20 nanometers and it is present between the outer and the inner membrane of the chloroplast.
Inner membrane - The inner membrane of the chloroplast forms a border to the stroma. It regulates passage of materials in and out of the chloroplast. In addition of regulation activity, the fatty acids, lipids and carotenoids are synthesized in the inner chloroplast membrane.
Stroma
Stroma is a alkaline, aqueous fluid which is protein rich and is present within the inner membrane of the chloroplast. The space outside the thylakoid space is called the stroma. The chloroplast DNA chlroplast ribosomes and the thylakoid sytem, starch granules and many proteins are found floating around the stroma.
Thylakoid System
The thylakoid system is suspended in the stroma. The thylakoid system is a collection of membranous sacks called thylakoids. The chlorophyll is found in the thylakoids and is the sight for the process of light reactions of photosynthesis to happen. The thylakoids are arranged in stacks known as grana.
Each granum contains around 10-20 thylakoids.
Thylakoids are interconnected small sacks, the membranes of these thylakoids is the site for the light reactions of the photosynthesis to take place. The word 'thylakoid' is derived from the Greek word "thylakos" which means 'sack'.
Important protein complexes which carry out light reaction of photosynthesis are embedded in the membranes of the thylakoids. The Photosystem I and the Photosystem II are complexes that harvest light with chlorophyll and carotenoids, they absorb the light energy and use it to energize the electrons.
The molecules present in the thylakoid membrane use the electrons that are energized to pump hydrogen ions into the thylakoid space, this decrease the pH and become acidic in nature. A large protein complex known as the ATP synthase controls the concentration gradient of the hydrogen ions in the thylakoid spaceto generate ATP energy and the hydrogen ions flow back into the stroma.
Thylakoids are of two types - granal thylakoids and stromal thylakoids. Granal thylakoids are arranged in the grana are pancake shaped circular discs, which are about 300-600 nanometers in diameter. The stromal thylakoids are in contact with the stroma and are in the form of helicoid sheets.
The granal thylakoids contain only photosystem II protein complex, this allows them to stack tightly and form many granal layers wiht granal membrane. This structure increases stability and surface areafor the capture of light.
The photosystem I and ATP synthase protein complexes are present in the stroma. These protein complexes acts as spacers between the sheets of stromal thylakoids.
Chloroplast Function
Functions of chloroplast:
The chloroplast genome
Like mitochondria, chloroplasts contain their own genetic system, reflecting their evolutionary origins from photosynthetic bacteria. The genomes of chloroplasts are similar to those of mitochondria in that they consist of circular DNA molecules present in multiple copies per organelle. However, chloroplast genomes are larger and more complex than those of mitochondria, containing approximately 120 genes.
The chloroplast genomes of several plants have been completely sequenced, leading to the identification of many of the genes contained in the organelle DNAs. These chloroplast genes encode both RNAs and proteins involved in gene expression, as well as a variety of proteins that function in photosynthesis. Both the ribosomal and transfer RNAs used for translation of chloroplast mRNAs are encoded by the organelle genome. These include four rRNAs (23S, 16S, 5S, and 4.5S) and 30 tRNA species. In contrast to the smaller number of tRNAs encoded by the mitochondrial genome, the chloroplast tRNAs are sufficient to translate all the mRNA codons according to the universal genetic code. In addition to these RNAcomponents of the translation system, the chloroplast genome encodes about 20 ribosomal proteins, which represent approximately a third of the proteins of chloroplast ribosomes. Some subunits of RNA polymeraseare also encoded by chloroplasts, although additional RNA polymerase subunits and other factors needed for chloroplast gene expression are encoded in the nucleus.
Import and sorting of chloroplast proteins
Protein import into chloroplasts generally resembles mitochondrial protein import. Proteins are targeted for import into chloroplasts by N-terminal sequences of 30 to 100 amino acids, called transit peptides, which direct protein translocation across the two membranes of the chloroplast envelope and are then removed by proteolytic cleavage. As in mitochondria, molecular chaperones on both the cytosolic and stromal sides of the envelope are required for protein import, which requires energy in the form of ATP. In contrast to the pre-sequences of mitochondrial import, however, transit peptides are not positively charged and the translocation of polypeptide chains into chloroplasts does not require an electric potential across the membrane.
Protein import into the chloroplast stroma: Proteins are targeted for import into chloroplasts by a transit peptide at their amino terminus. The transit peptide directs polypeptide translocation through the Toc complex in the chloroplast outer membrane. Proteins incorporated into the thylakoid lumen are transported to their destination in two steps. They are first imported into the stroma, as already described, and are then targeted for translocation across the thylakoid membrane by a second hydrophobicsignal sequence, which is exposed following cleavage of the transit peptide. The hydrophobic signal sequence directs translocation of the polypeptide across the thylakoid membrane and is finally removed by a second proteolytic cleavage within the lumen.
Within the thylakoid membranes of the chloroplast, are two photosystems. Photosystem I optimally absorbs photons of a wavelength of 700 nm. Photosystem II optimally absorbs photons of a wavelength of 680 nm. The numbers indicate the order in which the photosystems were discovered, not the order of electron transfer. Under normal conditions electrons flow from PSII through cytochrome bf (a membrane bound protein analogous to Complex III of the mitochondrial electron transport chain) to PSI. Photosystem II uses light energy to oxidize two molecules of water into one molecule of molecular oxygen. The 4 electrons removed from the water molecules are transferred by an electron transport chain to ultimately reduce 2NADP+ to 2NADPH. During the electron transport process a proton gradient is generated across the thylakoid membrane. This proton motive force is then used to drive the synthesis of ATP. This process requires PSI, PSII, cytochrome bf, ferredoxin-NADP+ reductase and chloroplast ATP synthase. I. Photosystem II Photosystem II transfers electrons from water to plastoquinone and in the process generates a pH gradient. Plastoquinone (PQ) carries the electrons from PSII to the cytochrome bf complex. Plastoquinone is an analog of Coenzyme Q. The only differences are the methyl groups replacing the methoxy groups of Q and a variable isoprenoid tail. Plastoquinone can functions as a one or two electron acceptor and donor. When it is fully reduced to PQH2 it is called plastoquinol. Like CoQ, PQ is a lipophilic mobile electron carrier carrying electrons from PSII to cytochrome bf. Photosystem II is homologous to the purple bacterial photoreaction center we talked about previously. PSII is an integral membrane protein. The core of this membrane protein is formed by two subunits D1 and D2. These two subunits span the membrane and are homologous to subunits L and M of the bacterial photosystem. Of course PSII is more complicated than its prokaryotic counterpart. PSII contains a lot more subunits and additional chlorophylls to achieve a lot higher efficiency than bacterial systems. The overall reaction of PSII is shown below. 2PQ + 2H2O O2 +2PQH2 O O H3C H3C CH3 C H2 C H C C H2 CH3 H n = 6-10 Plastoquinone OH OH H3C H3C CH3 C H2 C H C C H2 CH3 H n Plastoquinol 2e- 2H+ + The ribbon diagram of the crystal structure of PSII is shown below. The D1 subunit is shown in red and is homologous to the L subunit of the bacterial photosystem. The D2 subunit is shown in blue and is homologous to the M subunit of the bacterial photosystem. The structure of a bound cytochrome molecule is shown in yellow. The chlorophyll molecules are shown in green. The manganese center is shown in purple. Just as in the bacterial photosystem, there is a special pair of chlorophylls in PSII bound by D1 and D2 that are in close proximity of each other. This special pair is analogous to the special pair of bacteriochlorophylls in the bacterial photosystem. The PSII special pair consists of 2 chlorophyll a molecules that absorb light at an optimal wavelength of 680 nm. This special pair of chlorophylls is called P680. On excitation-either by the absorption of a photon or exciton transfer-P680* rapidly transfers an electron to a nearby pheophytin a. Pheophytin a is a chlorophyll a molecule with the Magnesium replaced by two protons. The electron is then transferred to a tightly bound plastoquinone at the QA site. The electron is then transferred to an exchangeable plastoquinone located at the QB site of the D2 subunit. The arrival of a second electron to the QB site with the uptake of two protons from the stroma produces plastoquinol, PQH2. When the electron is rapidly transferred from P680* to pheophytin a, a positive charge is formed on the special pair, P680.+. P680+ is an incredibly strong oxidant which N N H N H N H3C H3C HC CH2 CH3 CH2 H3C O H O H3C O O P O Pheophytin a N N N N H3C H3C HC CH2 CH3 CH2 H3C O H O H3C O O P O Chlorophyll a Mg extracts electrons from water molecules bound at the manganese center. The structure of this manganese center includes 4 Manganese ions, a calcium ion, a chloride ion, and a tyrosine radical. Manganese is the core of this redox center because it has four stable oxidation states (Mn2+, Mn3+,Mn4+ and Mn5+) and coordinates tightly to oxygen containing species. Each time the P680 is excited and an electron is kicked out, the positively charged special pair extracts an electron from the manganese center. 2H2O O2 + 4e- 4 electrons must be transferred to 2 molecules of plastoquinone in order to oxidize H2O to molecular oxygen. This requires 4 photochemical steps. The Manganese center is oxidized one electron at a time, until two molecules of H2O are linked to form O2 which is then released from the center. A tyrosine residue not shown participates in the proton electron transfers. The structures are designated S0 through S4 to indicated the number of electrons removed. The oscillation in O2 evolution dampens over repeated flashes and converges to an average value. We know the manganese center exists in five different oxidation states numbered for S0 to S4 as shown above. One electron and a proton are removed during each photochemical step. When S4 is attained, an O2 molecule is released and two new molecules of water bind. The reason the third pulse of light produces O2 is because the resting state of the PSII in the chloroplast is S1 not S0. PSII spans the thylakoid membrane. The site of plastoquinone reduction is on the stroma side of the membrane. The manganese complex is on the thylakoid lumen side of the membrane. For every four electrons harvested from H2O, 2 molecules of PQH2 are formed extracting four protons from the stroma. The four protons formed during the oxidation of water are released into the thylakoid lumen. This distribution of protons across the thylakoid membrane generate a pH gradient with a low pH in the lumen and a high pH in the stroma. II. Cytochrome bf The plastoquinol formed by PSII contributes its electrons through an electron transport chain that terminates at PSI. The intermediary electron transfer complex between PSII and PSI is cytochrome bf also known as cytochrome b6f. In this electron transfer complex electrons are passed one at a time from plastoquinol to plastocyanin (Pc) a copper protein of the thylakoid lumen. The reaction is shown below: PQH2 +2Pc(Cu2+) 2Pc(Cu+ ) + 2H+ The protons are released into the thylakoid lumen. Plastocyanin is a water soluble electron carrier found in the thylakoid lumen of chloroplasts. It contains a single Copper atom coordinated to two histidine residues and a cysteine residue in a distorted tetrahedron. The molecule is intensely blue in the cupric form. This mobile electron carrier carries electrons from cytochrome bf to PSI. The electron transfer of cytochrome bf is very similar to the electron transfer catalyzed by Complex III of the mitochondrian. The subunit components of cytochrome bf are homologous to the subunits of Complex III, cytochrome c reductase. The cytochrome bf contains two b-type heme cytochromes, a Reiske protein-type Fe-S protein, and a ctype cytochrome similar to cytochrome c1. This enzyme transfers electrons from plastoquinol through the same Q cycle as Complex III. The net result is two protons are picked up from the stroma side of the thylakoid membrane and 4 protons are released into the lumen contributing to the pH gradient. III. Photosystem I. The final stage of the light reactions is catalyzed by PSI. This protein has two main components forming its core, psaA and psaB. These two subunits are quite a bit larger that the core components of PSII and the bacterial photosystem. Nonetheless, the subunits are all homologous. The psaA and psaB subunits are shown in yellow with the regions homologous to the core of PSII shown in red and blue. Chlorophyll molecules are shown in green and the 3 4Fe-4S clusters are also shown in green. A special pair of chlorophyll a molecules lies at the center of the structure which absorbs light maximally at 700 nm. This special pair is denoted P700. Upon excitation-either by direct absorption of a photon or exciton transfer- P700* transfers an electron through a chlorophyll and a bound quinone (QA) to a set of 4Fe-4S clusters. From these clusters the electron is transferred to ferredoxin (Fd) a water soluble mobile electron carrier located in the stroma which contains a 2Fe-2S cluster coordinated to 4 cysteine residues. The electron transfer produces a positive charge on the special pair which is neutralized by the transfer of an electron from a reduced plastocyanin. The overall reaction is shown below. Pc(Cu+ ) + Fdox Pc(Cu2+) + Fdred The structure of ferredoxin is shown to the left. Ferredoxin contains a 2Fe-2S cluster which accepts electrons from PSI and carries them to ferredoxin-NADP+ reductase. Pc(Cu2+) + e- Pc(Cu+ ) Eo ’ = +0.37 V Fdox + e- Fdred Eo ’ = -0.45 V The electron acceptor in the overall reaction shown above is the oxidized ferredoxin, the electron donor is the reduced plastocyanin. From the reduction potentials listed above, the change in reduction potential is: ∆ Eo ’ = -0.45 - 0.37 = -0.82 V which corresponds to a ∆ Go ’ = 79.1 kJ/mol, very endergonic. This uphill electron transfer is driven the by absorption of a 700-nm photon of light which has an energy of 171 kJ/mol. The electron transport pathway between PSII and PSI is called the Z-scheme because the redox diagram looks like a sideways letter Z. IV. Ferredoxin-NADP+ Reductase Ferredoxin is a strong reductant but can only function in one electron reductions. NADP+ can only accept 2 electrons in the form of a hydride. Clearly we need an intermediary to facilitate the electron transfer. The transfer of electrons from reduced ferredoxin to NADP+ it catalyzed by ferredoxin-NADP+ reductase which is flavoprotein. This complex contains a tightly bound FAD which accepts the electrons one at a time from ferredoxin. The FADH2 then transfers a hydride to NADP+ to form NADPH. This reaction takes place on the stromal side of the thylakoid membrane. The uptake of a proton by NADP+ further contributes to the pH gradient across the thylakoid membrane. V. Chloroplast ATP Synthase The transport of electrons from water to NADP+ generated a pH gradient across the thylakoid membrane. This proton motive force is used to drive the synthesis of ATP. The synthesis of ATP in the chloroplast is nearly identical with ATP synthesis in the mitochondria. The pH gradient generated between the stroma and the thylakoid lumen is possible because the thylakoid membrane is impermeable to protons. When the chloroplast is illuminated the thylakoid lumen becomes markedly acidic, pH ≈ 4. The pH of the stroma is around 7.5. The light induced pH gradient is about 3.5 pH units. The transmembrane electrical potential is not a significant factor in the proton motive force in the chloroplast because the thylakoid membrane is permeable to Cl- and Mg2+. Because of this permeability, the thylakoid lumen remains electrically neutral while the pH gradient is generated. A pH gradient of 3.5 pH units thus corresponds to a proton motive force of -20 kJ/mol. The ATP synthase of the chloroplast is called the CF1-CF0 complex where C stands for chloroplast and F1 and F0 relate to the homologous ATP synthase of the mitochondria. The mitochondrial and the chloroplast ATP synthase are essentially identical with similar subunits and subunit stoichiometries. The catalytic subunit is the β subunit of CF1. The CF1 complex lies in the stroma. The CF0 complex channels protons from the thylakoid lumen to the stroma driving rotation of the 12 c subunits which in turn drives ATP synthesis. The ATP formed is released into the stroma where it is needed for the dark reactions of photosynthesis. VI. Cyclic Photophosphorylation There is an alternative pathway for the electrons arising from PSI giving photosynthesis versatility. The electrons carried in reduced ferredoxin can be transferred to the cytochrome bf complex rather than the ferredoxin-NADP+ reductase complex as shown below. The electrons then flow back through cytochrome bf to reduce plastocyanin, which then reduces the P700+ to complete the cycle. The net outcome of this cyclic flow of electrons is the pumping of protons across the thylakoid membrane by the cytochrome bf complex, producing a pH gradient which then drives the synthesis of ATP. This process produces ATP without NADPH generation. In addition PSII does not participate in cyclic photophosphorylation, so O2 is not generated during this process. Cyclic photophosphorylation only occurs when the NADP+ concentration becomes limiting, such is the case when there is a very high ratio of NADPH/NADP .
Nutrients and Minerals involved in Chloroplast Development
Manganese Manganese is present in most Fe-Mg containing igneous rocks and when solubilized by weathering, forms several secondary minerals mostly pyrolusite (MnOz) and manganite (MnOOH). Thus, most soil Mn is insoluble and not available to plant roots. The plant-available form of free Mn in soil solution is the divalent cation Mn2+ . Manganese solubility is very much dependent on soil pH, decreasing 100 fold with each unit increase in pH. Consequently Mn is much more soluble and available to plants in acid soils (pH < > Mn2+ + 2H20 Here, minerals containing Mn in oxidized form (Mn3+ or Mn4+) will be reduced to Mn2+ by acquiring electrons (e") through the microbial oxidation of organic carbon. To meet the nutritional needs of plants for Mn, the soil solution and exchangeable Mn should be 2-3 ppm and 0.2-5.0 ppm, respectively. In acid soils, soluble Mn may exceed these levels by substantial amounts and become toxic to plants. This is likely in situations where soil minerals contain Mn, the organic matter content is high, the pH is low and the soil is periodically waterlogged (low in free oxygen). Under such conditions, acid inhibition of plant growth 4 Liqht Photons Photosynthesis Fig. 2. Oxygen evolving mechanism of plant photosynthesis in which water repaces electrons driven by light from chlorophyll through the re-dox action of Mn. may be due primarily to toxic levels of available Mn. Under opposite conditions (parent minerals low in Mn, alkaline pH, low organic matter and good drainage) inadequate supplies of available Mn are likely. Some 13 million acres in 30 states of the US exhibit chronically inadequate levels of Mn. For the turf manager, Mn insufficiency is a more likely problem than its toxicity. Soluble soil Mn is also present in the form of Mn-chelates. These organic chelates are excreted from plant roots or produced during the metabolism of organic residues. They will bind with Mn2+ but remain in solution as uncharged molecules. Much of the soluble Mn in organic soils will be present in a chelated form. Being soluble, Mn chelates are mobile in the soil and can be drawn to roots via mass flow when plants are removing water from the soil through transpiration. This process can assist in Mn uptake as we will see below. Manganese uptake Plant roots can absorb Mn primarily as the divalent cation (Mn2+). It enters root cells by crossing their plasma membrane via a TABLE 2. MANGANESE FUNCTIONS IN HIGHER PLANTS INCLUDING TURFGRASSES Essential component of two enzymes: • 02 evolving component of photosynthesis (PS II) • Mn-containing superoxide dismutase (MnSOD) Activating cofactor for -3 5 enzymes required for: • Respiratory metabolism of organic acids (TCA cycle) • RNA synthesis in chloroplasts (RNA polymerase) • Shikimic acid pathway (aromatic amino acid synthesis) • Lignin synthesis in roots (cell wall peroxidases) • Lipid synthesis including chlorophyll and carotenoids • Regulating auxin levels and isoprenoid synthesis (GA) Required for root elongation and lateral root formation specific transporter protein following an electrical gradient (cell interior is more negative than the cell wall). Other divalent micronutrient cations, such as Copper (Cu2+) and Zinc (Zn2+), do not compete with Mn2+ for membrane transport sites although they do compete with each other (Bowen 1969). However, the abundance of several macronutrients on cation exchange sites within the cell walls of root cells (the apoplasm) can influence Mn absorption by roots. Manganese is much less available from soils of high pH. This is in large part due to the high concentrations of Calcium and Magnesium ions (Ca2+ & Mg2+) in such soils. When Ca2+ and Mg2+ dominate cation exchange sites in root cell walls, there are few places for less abundant ions like Mn2+ to be retained. Since cations absorbed by roots are drawn mostly from those present within the cell wall matrix, any less abundant ions (including most micronutrients) will have a difficult time reaching the plasma membrane when they are vastly outnumbered by basic divalent cations. This is the reason why most micronutrient metals are less available in soils of neutral or alkaline pH. In acid soils, the principle competing cation is Hydrogen (H+ ) and it is not held on exchange sites as tightly as most divalent cations. However, if other cations such as aluminum (Al3+), potassium (K+ ) or other micronutrients greatly outnumber Mn2+ even in acid soils, Mn may become deficient. Even so, the most common method for increasing Mn availability to plants is to apply acid fertilizers such as (NH4)2S04 or urea. The availability and absorption of Mn from soils is more influenced by microbial activity than is any other. Most soil Mn is insoluble and not available to plant roots. To be absorbed by plants, Mn must be reduced to the divalent Mn2+ ion and this requires the action of microbial metabolism (Marschner 1995). Under acid conditions, Mn-containing minerals will slowly be solubilized and several ionic To meet the nutritional needs of plants for Mn, the soil solution and exchangeable Mn should be 2-3 ppm and 0.2-5.0 ppm, respectively. acids and phenolics) to the soil immediately adjacent to the roots (rhizosphere). These exudates along with dead root cells (root cap cells, root hairs, epidermal and cortical cells) fuel microbial activity and, especially when the soil is waterlogged, supply electrons to reduce Mn ions to the plant-available Mn2+ form. This ion can then be absorbed by root cells as described above. In grasses, there is a cooperative mechanism for acquiring Mn by plants experiencing an Iron (Fe) deficiency. Because of its low solubility and the inability of membrane transporters to accommodate trivalent ions, Fe3+ poses some special problems in its availability to plants. Grasses have a unique strategy for